Research
Table of contents
Cavity-enhanced two-dimensional infrared spectroscopy of gas-phase molecules
Cavity-enhanced two-dimensional infrared spectroscopy (CE-2DIR) is a technique proposed by Allison in 20171, which will enable several orders of magnitude enhancement of sensitivity in 2DIR spectroscopy. While similar to cavity-enhanced transient absorption spectroscopy2, CE-2DIR has not been demonstrated yet.
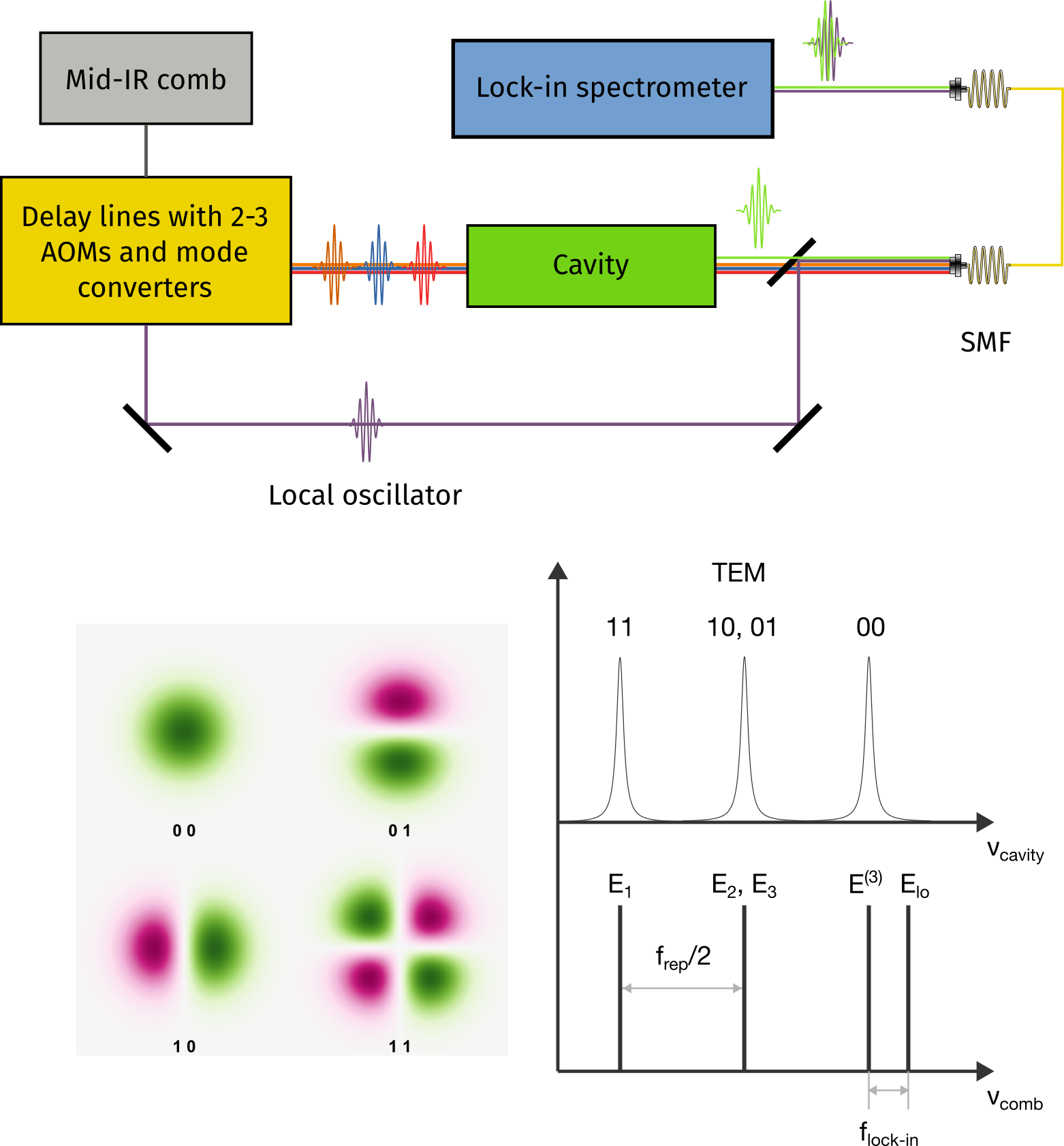
We will use our home-made Yb:fiber oscillator and a mid-infrared optical parametric oscillator as the seed comb as seen in the figure above. Delay lines will split the original pulse train into 4 separate pulse trains. Placing acousto-optic modulators and mode converters into appropriate delay line branches will match the transverse spatial profiles and offset frequencies of optical frequency comb beams to higher order cavity modes. The spatial profile of the third-order nonlinear signal will be determined by the product of transverse cavity mode profiles. Adjusting time-ordering of the pulses will make either the rephasing or nonrepashing signal resonant with the fundamental Gaussian mode. The combined signal and the local oscillator beams will pass through a spatial filter (a single-mode fiber) and their heterodyne beat at RF frequencies will be demodulated to obtain the 2DIR spectrum at noise levels close to the shot noise limit.
High resolution 2DIR gas-phase spectroscopy will have important scientific applications. It will enable us to directly measure the thermalization rate of the nonequilibrium velocity distribution of rovibrational (infrared) coherences in the gas phase. Dynamics of this distribution affect the appearance of IR linear spectra through collisional narrowing of absorption lines (the Dicke effect) and other effects of velocity-changing collisions3. Moreover, hi-res 2DIR spectroscopy is a perfect tool to measure the collisional transfer of population, which presents itself as the line mixing phenomenon in linear spectra. High resolution 2DIR gas-phase spectroscopy also has the potential to serve as a tool to quantify composition of gas samples with better selectivity than linear gas-phase spectroscopy. Compared to diagonal or linear response, the off-diagonal 2DIR response of different species overlaps to a lesser degree. This feature of 2DIR spectroscopy will make it easier to quantify concentrations in complex gas mixtures. The selectivity of 2DIR gas-phase spectroscopy can be further enhanced as we described in the following section.
Polarization control of 2DIR gas-phase spectra
We have developed a theory and a Python library to describe the polarization dependence of 2DIR gas-phase spectra4. Using these tools we discovered new polarization conditions specific to the gas phase, which allow us to selectively suppress subsets of third-order pathways in 2DIR spectra. Assuming separation of rotational and vibrational degrees of freedom, all third-order nonlinear pathway amplitudes can be separated into 7 distinct classes (\(\sigma_i\))5 with regards to their dependence on pulse polarizations. The general formula is given by:
\[ \begin{split} R^{(0)}_{0}(\boldsymbol{\epsilon}; \mathbf{J}) =& \frac{c_{00}^{\sigma_i}}{60(2J_{i}+1)^{3/2}}\big( c_{12}^{\sigma_i} \cos(\theta_{1}+\theta_{2}-\theta_{3}-\theta_{4})\\ &+c_{13}^{\sigma_i} \cos(\theta_{1}-\theta_{2}+\theta_{3}-\theta_{4})\\ &+c_{14}^{\sigma_i} \cos(\theta_{1}-\theta_{2}-\theta_{3}+\theta_{4}) \big). \end{split} \]Here \(\theta_{i} \) are linear polarization angles of three pulses and the detection angle. Each class is associated with a set of four coefficients (\(c_{00}^{\sigma_i}, c_{12}^{\sigma_i}, c_{13}^{\sigma_i}, c_{14}^{\sigma_i}\)). This decomposition of the total response is illustrated below with non-rephasing 2DIR spectra of methyl chloride C-Cl stretching mode.
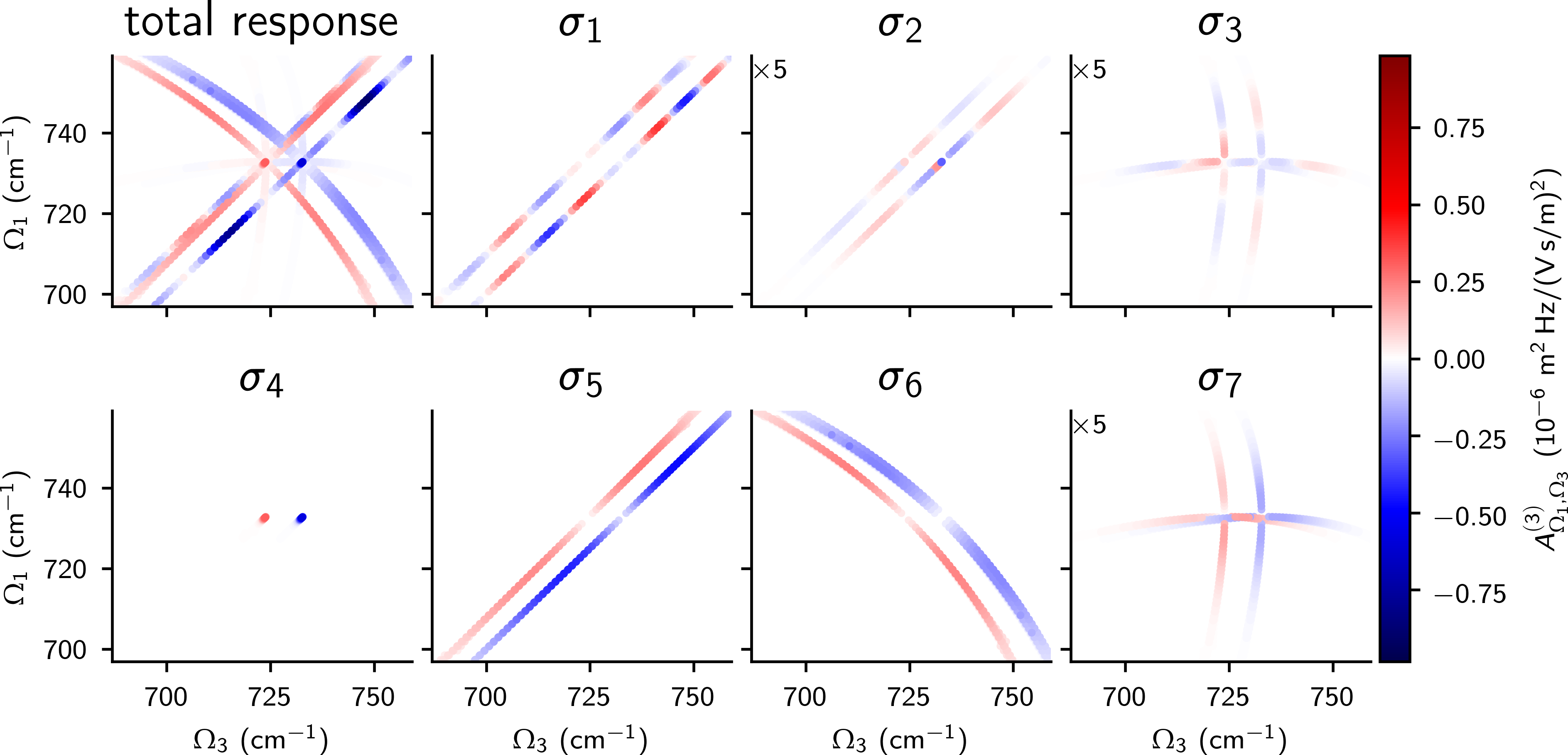
Knowing the polarization dependence, we presented polarization conditions that suppress parts of the total molecular response. Figure below demonstrates four of these conditions with non-rephasing methyl chloride spectra. The table below gives the amplitudes of \(R\)-factors for each polarization sequence and for each class, which explains the appearance of the spectra.
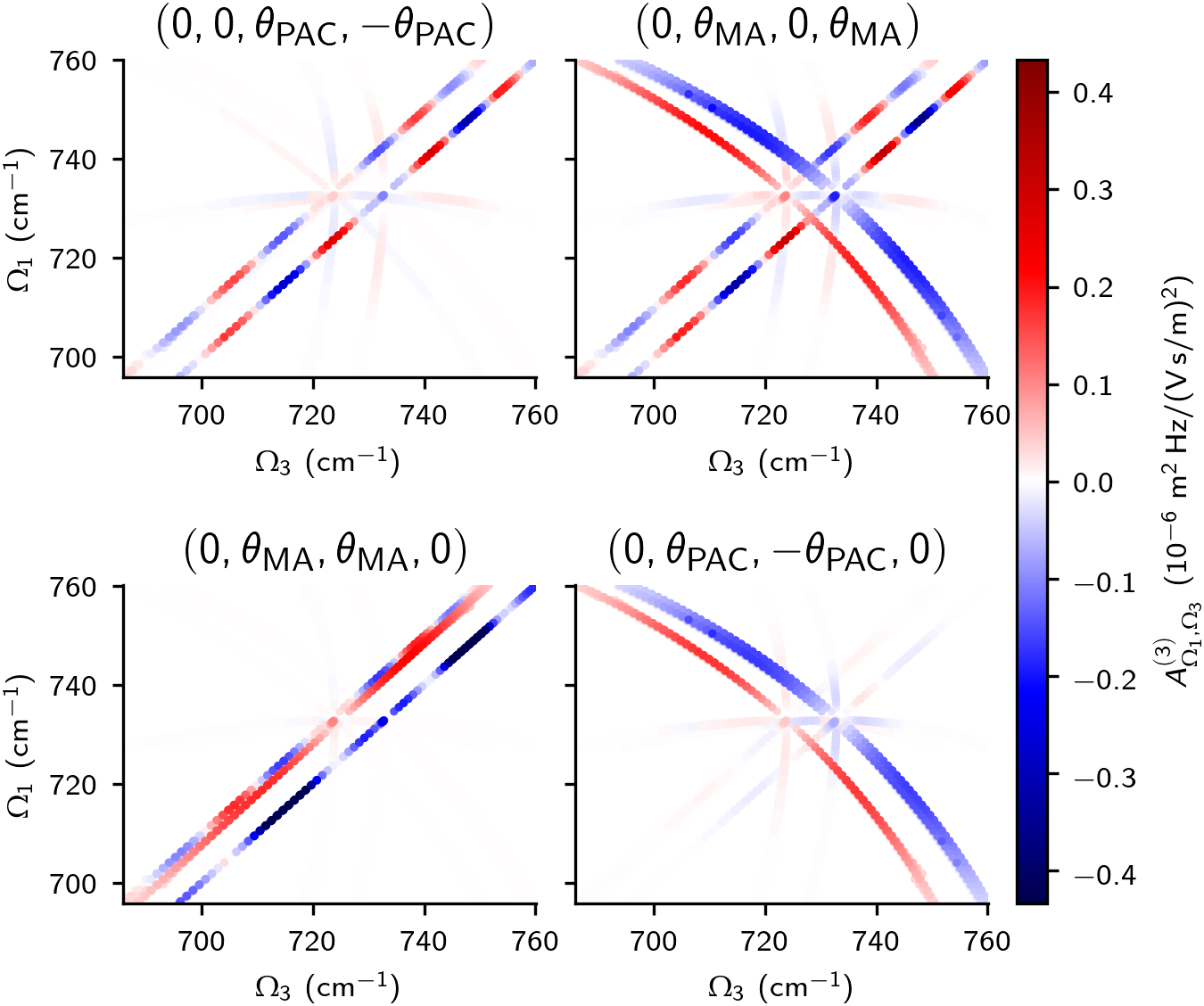
Sequence | \(\sigma_1\) | \(\sigma_2\) | \(\sigma_3\) | \(\sigma_4\) | \(\sigma_5\) | \(\sigma_6\) | \(\sigma_7\) |
---|---|---|---|---|---|---|---|
(0, MA, 0, MA) | 1/9 | 0 | -1/9 | 1/9 | 0 | 1/9 | 0 |
(0, 0, PAC, -PAC) | 2/21 | -1/21 | -1/21 | 1/21 | 0 | 0 | 1/21 |
(0, MA, MA, 0) | 1/9 | -1/9 | 0 | 1/9 | 1/9 | 0 | 0 |
(0, PAC, -PAC, 0) | 0 | 1/21 | -1/21 | 1/21 | 0 | 2/21 | -1/21 |
Acknowledgments
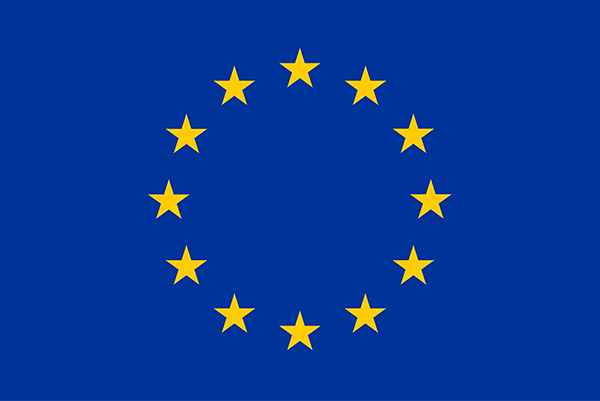
This project receives funding from the European Union’s Horizon 2020 research and innovation programme under the Marie Skłodowska-Curie grant agreement No 101028278.
-
T. K. Allison, “Cavity-enhanced ultrafast two-dimensional spectroscopy using higher order modes,” Journal of Physics B: Atomic, Molecular and Optical Physics, vol. 50, no. 4, p. 044004, 2017, doi: 10.1088/1361-6455/50/4/044004. ↩︎
-
M. A. R. Reber, Y. Chen, and T. K. Allison, “Cavity-enhanced ultrafast spectroscopy: Ultrafast meets ultrasensitive,” Optica, vol. 3, no. 3, p. 311, Mar. 2016, doi: 10.1364/OPTICA.3.000311;
M. Silfies et al., “Widely tunable cavity-enhanced frequency combs,” Optics Letters, vol. 45, no. 7, pp. 2123–2126, 2020, doi: 10.1364/OL.389412;
M. C. Silfies, G. Kowzan, N. Lewis, and T. K. Allison, “Broadband cavity-enhanced ultrafast spectroscopy,” Physical Chemistry Chemical Physics, vol. 23, no. 16, pp. 9743–9752, 2021, doi: 10.1039/D1CP00631B;
M. C. Silfies, A. Mehmood, G. Kowzan, E. G. Hohenstein, B. G. Levine, and T. K. Allison, “Ultrafast internal conversion and photochromism in gas-phase salicylideneaniline,” The Journal of Chemical Physics, vol. 159, no. 10, p. 104304, Sep. 2023, doi: 10.1063/5.0161238. ↩︎ -
G. Kowzan, P. Wcisło, M. Słowiński, P. Masłowski, A. Viel, and F. Thibault, “Fully quantum calculations of the line-shape parameters for the Hartmann-Tran profile: a CO-Ar case study,” Journal of Quantitative Spectroscopy and Radiative Transfer, vol. 243, p. 106803, 2020, doi: 10.1016/j.jqsrt.2019.106803;
G. Kowzan et al., “Subpercent agreement between ab initio and experimental collision-induced line shapes of carbon monoxide perturbed by argon,” Physical Review A, vol. 102, no. 1, p. 012821, 2020, doi: 10.1103/physreva.102.012821.
J.-M. Hartmann, C. Boulet, and D. Robert, Collisional effects on molecular spectra. Laboratory experiments and models, consequences for applications. Amsterdam: Elsevier, 2008. ↩︎ -
G. Kowzan and T. K. Allison, “Theory of rotationally resolved two-dimensional infrared spectroscopy including polarization dependence and rotational coherence dynamics,” Phys. Rev. A, vol. 106, no. 4, p. 042819, Oct. 2022, doi: 10.1103/PhysRevA.106.042819.
G. Kowzan and T. K. Allison, “Controlling Rotationally Resolved Two-Dimensional Infrared Spectra with Polarization,” J. Phys. Chem. Lett., vol. 13, no. 50, pp. 11650–11654, Dec. 2022, doi: 10.1021/acs.jpclett.2c03331.
G. Kowzan, “rotsim2d
: Simulate 2D rovibrational spectra of gas-phase molecular samples. Zenodo. https://doi.org/10.5281/zenodo.6654257.” Zenodo, Jun. 2022. doi: 10.5281/zenodo.6654257. ↩︎ -
In the cited papers these classes were labeled with capital \(\Theta_i\) letters, which could be confused with linear polarization angles labeled with small \(\theta_i\) letters. ↩︎